The Cold Molecule Group
Why cool atoms and molecules to low temperatures?
Our experiments start by producing a molecular beam of the species of interest. When a gas expands through a small orifice into an evacuated chamber at very low pressure, it forms a directed beam of particles moving at nearly the same speed. The density of the molecules is typically very low and there are only few collisions between them. This technique has been used for over 100 years to study atoms and molecules and the interaction of the particles with electric, magnetic and electromagnetic fields. We can slow the beam to standstill using electric fields or precisely tuned lasers. The molecules can then be trapped and cooled to very low temperatures of a few millionths of a degree above absolute zero. At such a low temperature the molecules move with a speed of about 1 cm/s, compared to 300 m/s at room temperature. This allows us to study the molecules, the interactions between the molecules and the interaction of light with the molecules with incredible precision.
Using lasers to reduce the thermal motion of particles

For over 30 years atoms have been trappend an cooled in a magneto-optical trap (MOT) using a combination of precisely tuned lasers and magnetic fields. The MOT has enabled the invention of precise instruments, such as atomic clocks, precise GPS, magnetometers, gravimeters and accelerometers. It has also enabled new fundamental research with unprecedented precision and the study of matter dominated by quantum effects. Molecules offer even more; they are not spherically symmetric and can be oriented, they can rotate, vibrate, and have an electric and/or a magnetic dipole moment. Precisely controlled molecules can be used to test the most fundamental models in physics, to study new phases of matter, to model complex quantum systems and can serve as elements of a scalable quantum processor. However, the higher complexity makes it more difficult to cool the molecules.
The MOT relies on the continuous scattering of photons, i.e. excitation of the particle followed by spontaneous emission. Atoms can scatter millions of photons by choosing a closed optical cycle between two electronic states.
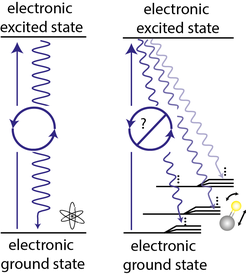
Molecules are more difficult to laser-cool because the laser can excite rotational and vibrational degrees of freedom. After a few photon-scattering events the molecule will end up in a "dark" state that is not coupled to the laser light and the optical cycle is broken.
The spontaneous emission to dark rotational states can be prevented by using quantum mechanical selection rules. However, there are no selection rules to prevent the emission to dark vibrational states. By selecting a molecule whose bond is not affected by promoting an electron into a higher orbital with a laser it is possible to minimize the probability of exciting vibrations. The periodic table offers 6903 hetero-nuclear diatomic molecules, so there are plenty of options to choose from. Currently, our favorite molecule for laser cooling is aluminum monofluoride (AlF). With only one laser to address a small 'leak' to a vibrationally excited state we are able to scatter over 10000 photons, enough to slow, trap and cool the molecules.
.Aluminum monofluoride (AlF) - a great molecule for laser cooling
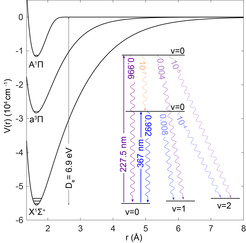
Most applications of ultracold molecules require and asymmetric charge distribution, i.e., an electric dipole moment and therefore heteronuclear, polar molecules. To reach a high density it is useful to start with a stable molecule such as NO or CO, but these molecules are very difficult to laser cool, or do not have a dipole moment. AlF cannot be purchased in a gas bottle, but can be produced with a very high efficiency using the reaction 2Al + AlF3 → 3 AlF at about 1000 ℃. It can be laser-cooled to very low temperatures and has a large electric dipole moment of 1.5 Debye in the electronic ground state.
It has a very strong transition in the UV (excited state lifetime of 2 ns) and the probability of exciting a vibrational mode is extremely low. This allows exerting a large optical force which results in an exceptionally large capture velocity for the MOT, only limited by the currently available laser power in the UV. This means that we can build the MOT close to the molecular source, where the density is still very high and capture a large fraction of the molecular beam. For molecule standards the MOT is relatively simple. There is no need to modulate the laser frequency to address unresolved hyperfine structure in the electronic ground-state and polarization modulation is not required to destabilize dark hyperfine states.
AlF has a metastable (a3Π) state that lies in between the ground and first excited singlet states. The transition to this state is nominally forbidden, but becomes weakly allowed due to spin-orbit mixing with the singlet states. Laser cooling on such weakly-allowed intercombination lines are commonly used to cool atoms to a very low temperature at a very high density.
Cryogenic buffergas molecular beam
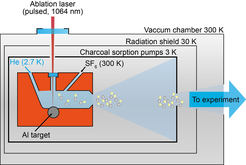
One method to produce a beam of cold, slow-moving molecules is buffer gas cooling. In most cases the molecule of interest must be produced in the gas-phase. This can be an oven to heat up precursor material, by ablating material using a pulsed laser or by dissociating larger molecules. The hot molecules are then cooled via collisions with a cryogenic buffer gas (helium, neon) inside a copper cell that is connected to a closed-cycle refrigerator and keeps the temperature as low as 2.7 K. The molecules cool rapidly and a molecular beam is extracted through a small orifice in the cell.
To produce AlF, we laser-ablate an Al rod in the presence of a continuous stream of 3 K helium mixed with a small amount of 300 K SF6. The reaction of the hot Al atoms with SF6, NF3 and F2 has been shown to produce predominantly AlF molecules. This produces a highly intense, pulsed beam of molecules. We are currently investigating new possibilities to produce a, cold, bright and slow, continuous beam of AlF, by using an oven in combination with buffer gas cooling.
Spectroscopy first
We have currently two molecular beam machines running (see pictures below). One is a buffer gas molecular beam machine to investigate the molecule production and the optical cycling scheme (a). The second one is a standard supersonic beam machine to perform precise rf, microwave and optical spectroscopy (b). The molecules can be detected via laser-induced fluorescence or via state-selective ionization followed by mass-selective ion-detection on a micro-channel plate detector.
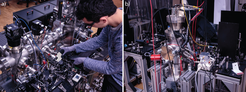
Our buffer gas molecular beam setup produces about 2x1012 molecules per steradian per pulse in the electronic, vibrational and rotational ground state. This is a good starting point for first laser-cooling experiments. To detect the molecules we lock a UV laser to a transition frequency in AlF, cross the laser beam with the molecular beam and image the emitted fluorescence onto a photo-multiplier tube (PMT). This is shown in part (a) of the figure below. Part (a) also shows that the buffer gas beam is much more intense than a more conventional supersonic beam, whose velocity is also 4 times higher.
We can also scan the laser frequency and record the fluorescence signal as a function of the laser frequency. The resulting Q-branch spectrum is shown in part (b) of the figure below. All Q-lines can be used for laser cooling.
A picture of the buffer-gas molecular beam source is shown in part (c) of the figure below.

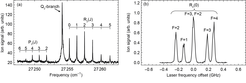
Optical cycling
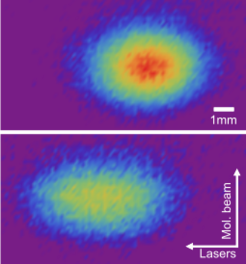
We next investigated optical cycling on the Q-lines of the A-X band in AlF and compared the experiment to a theoretical model. These experiments were successful in demonstrating that:
An AlF molecule can scatter about 210 photons before loss to the first vibrationally excited state, from which it can be efficiently recovered with a repump laser.
The hyperfine structure of the molecule allows rapid and continuous photon scattering without the formation of hyperfine 'dark states'.
Multiple Q-lines of the A-X band are suitable for optical cycling, as predicted by quantum mechanical selection rules.
Optical deflection on the A-X transition can generate transverse accelerations as high as 870 km/s2.
Laser cooling AlF molecules
The next step is to investigate the laser cooling on the Q-lines of the A-X band in AlF and compare the experiment to a theoretical model. From our spectroscopic investigations we conclude that AlF is an excellent candidate for laser-cooling:- Simple MOT. Compared to current molecule cooling experiments the AlF MOT is relatively simple to implement.
- Large capture velocity. A high photon scattering rate and a large photon recoil results in an exceptionally large capture velocity of the AlF MOT.
- Narrow-line cooling. Cooling the molecules on a spin-forbidden transition similar to Yb, Sr, Cd, etc. can lead to a large increase in the density at ultra-low temperatures