Catalysis with Oxides
Groupleader: Annette Trunschke
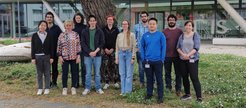
Motivation
The chemical diversity of metal oxides accounts for the importance of this materials class in various functional applications. Oxides are used for example as chemical sensors, adsorbents, and catalysts in photo-, electro-, andthermalcatalysis. The current challenges in the energy, transportand chemistry sectorsrequire new, more stable and effective catalysts to reduce or replace fossil fuels by closing the carbon cycle. In addition, the improvement of existing technologiesin heterogeneous catalysis, for example the production of chemical intermediates and consumer products, is of vital importance in terms ofsustainable utilization of resources and CO2 mitigationconsidering the dimension of these applications.
Research areas
Our work is devoted to the investigation of metal oxides for activation of C-H, O-O, and C-O bonds. The oxidation of short-chain alkanes and oxygenates on the surface of bulk and supported V-based mixed oxides, perovskites, and alkaline earth metal oxides is studied with the aim to identify descriptors to predict selectivity.1-3 In the field of CO and CO2 activation, the influence of transi metal oxide promoters on the reactivity of metal nano -particles is investigated.4,5
Scientific challenges in catalysis with oxides
The materials chemistry of high-performance metal-oxide catalysts is particularly intricate, because, according to the present empirical knowledge, superior selectivity to the desired reaction product at acceptable conversion of the reacting molecules requires a certain level of catalyst complexity. Catalysis in general inherently involves dynamic interactions between catalyst and reaction medium that are modulated by transport phenomena at various length and time scales. The material chemistry of bulk oxides as well as supports needs to counterbalance structural and electronic surface changes to enable stable operation. In addition, physical and textural properties of the oxide catalyst need to be adapted to ensure heat and mass transport in an optimal way.
Experimental techniques

Structurally well-defined metal oxides are prepared by applying various techniques of synthetic inorganic chemistry with focus on hydrothermal synthesis. Automated synthesis reactors (Figure 1) have been developed and equipped with analytic tools to detect key synthesis parameters that control the functionality. Raman and UV/Vis spectroscopy are relevant spectroscopic techniques that provide information concerning the speciation under synthesis conditions.7 Mass spectrometry in combination with electrospray ionisation (ESI) ion mobility spectrometry−mass spectrometry (IMS−MS), infrared multiple photon dissociation (IRMPD) spectroscopy, and infrared action spectroscopy in helium nano -droplets has been used ex-situ in collaboration w ith the Department of Molecular Physics.8 The microstructure of the oxide catalysts is analysed by electron microscopy.9 I nteractions of substrate and probe molecules with the catalyst surface are studied by infrared spectroscopy and microcalorimetry.4,5,10-13 Various spectroscopic techniques are applied in operando to monitor the catalysts under working conditions.1-3,14 The interpretation of spectroscopic data is assisted by close collaboration with theory.15 Reaction networks in oxidation reactions have been analysed by the variation of operation par ameters over a wide range.13,16,17 Selected research highlights are summarized below.
Design concepts in oxidation catalysis
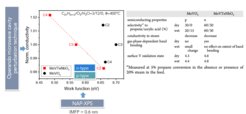
Monolayers of vanadium and molybdenum oxide supported on meso-porous silica SBA-15 were investigated to simulate the surface of mixed oxide catalysts.18 The workshows that the catalytic properties as consequence of the local electronic structure are by no means proportional to the loading or the dilution factor. Semiconductor oxides including n-type MoVTeNb-oxide, p-type MoV-oxide, n-type V2O5−x, and p-type vanadyl pyrophosphate (VPP) were investigated by operando conductivity and permittivity measurements complemented by near-ambient-pressure X-ray photoelectron spectroscopy.1,2 The results indicate that catalysts, which exhibit a high selectivity to the desired oxidation product at high alkane conversions, reveal the property of a “buffer” for charge carriers in their bulk that can be transported to the active sites when the delivery of redox equivalents between adsorbed molecules is too slow. Likewise, the surface layer containing the active sites is robust against chemical changes induced by the lack of redox equivalents leading to chemical oxidation or reduction of the sites. The change in the electronic structure of the surface is reflected in band bending, i.e., the formation of a dynamic (gas-phase-dependent) surface potential barrier that controls oxygen activation (Figure 2). Concepts solely based on ideal crystal structure motifs as functional active ensembles in oxidation catalysis are questioned by the investigation of a series of manganese tungstate catalysts that differ only in their particle morphology.3 The variable particle shape serves as a proxy that reflects the formation of varying abundance of redox active Mn2+/Mn3+ surface sites, which correlates with catalytic activity.The governing function of oxygen activation has also been observed in the oxidative coupling of methane (OCM) over alkaline earth oxides. Even though the bond dissociation energy of methane is large with 423 kJ/mol, the activation of oxygen is much more important to direct the selectivity towards the desired products.19 The strong basicity, which is needed for the reaction, is also a major weakness of those materials, forming stable hydroxides and carbonates. The latter are incapable of activating oxygen even at high temperatures.
References
- A. M. Wernbacher, P. Kube, M. Hävecker, R. Schlögl, and A. Trunschke, The Journal of Physical Chemistry C 123 , 13269 (2019)
- A. M. Wernbacher, M. Eichelbaum, T. Risse, S. Cap, A. Trunschke, and R. Schlögl, The Journal of Physical Chemistry C 123, 8005 (2019).
- X. Li, D. Teschner, V. Streibel, T. Lunkenbein, L. Masliuk, T. Fu, Y. Wang, T. Jones, F. Seitz, F. Girgsdies, F. Rosows ki, R. Schlögl, and A. Trunschke, Chemical Science 10, 2429 (2019).
- X. Huang, D. Teschner, M. Dimitrakopoulou, A. Fedorov, B. Frank, R. Kraehnert, F. Rosowski, H. Kaiser, S. Schunk, C. Kuretschka, R. Schlögl, M.-G. Willinger, and A. Trunschke , Angewandte Chemie International Edition 58 , 8709 (2019).
- M. Dimitrakopoulou, X. Huang, J. Kröhnert, D. Teschner, S. Praetz, C. Schlesiger, W. Malzer, C. Janke, E. Schwab, F. Rosowski, H. Kaiser, S. Schunk, R. Schlögl, and A. TrunschkeFaraday Discussions 208 , 207 (2018).
- J. Noack, F. Rosowski, R. Schlögl, and A. Trunschke, Zeitschrift für anorganische und allgemeine Chemie 640 , 2730 (2014).
- K. Wenderich, J. Noack, A. Kärgel, A. Trunschke, and G. Mul, European Journal of Inorganic Chemistry 2018 , 917 (2018).
- M. Marianski, J. Seo, E. Mucha, D. A. Thomas, S. Jung, R. Schlögl, G. Meijer, A. Trunschke, and G. von Helden, The Journal of Physical Chemistry C 123, 7845 (2019).
- L. Masliuk, M. Heggen, J. Noack, F. Girgsdies, A. Trunschke, K. E. Hermann, M. G. Willinge r, R. Schlögl, and T. Lunkenbein, The Journal of Physical Chemistry C 121 , 24093 (2017).
- P. Preikschas, J. Bauer, X. Huang, S. Yao, R. Naumann d'Alnoncourt, R. Kraehnert, A. Trunschke, F. Rosowski, and M. Driess, ChemCatChem 11 , 885 (2019).
- H. V. Le, S. Parishan, A. Sagaltchik, H. Ahi, A. Trunschke, R. Schomäcker, and A. Thomas, Chemistry – A European Journal 24 , 12592 (2018).
- B. Calvo, C. P. Marshall, T. Krahl, J. Kröhnert, A. Trunschke, G. Scholz, T. Braun, and E. Kemnitz, Dalton Transactions 47 , 16461 (2018).
- P. Kube, B. Frank, S. Wrabetz, J. Kröhnert, M. Hävecker, J. Velasco - Vélez,J. Noack, R. Schlögl, and A. Trunschke, ChemCatChem 9 , 573 (2017).
- A. Trunschke, et al., ACS Catalysis 7 , 3061 (2017).
- A. Kubas, J. Noak, A. Trunschke, R. Schlögl, F. Neese, and D. Maganas, Chemical Science 8 , 6338 (2017).
- C. Sprung, G. S. Yablon sky, R. Schlögl, and A. Trunschke, Catalysts 8, 330 (2018).
- P. Kube, B. Frank, R. Schlögl, and A. Trunschke, ChemCatChem 9 , 3446 (2017).
- T. Fu, Y. Wang, A. Wernbacher, R. Schlögl, and A. Trunschke, ACS Catalysis 9 , 4875 (2019).
- L. Thum, M. Rudolph , R. Schomäcker, Y. Wang, A. Tarasov, A. Trunschke, and R. Schlögl, The Journal of Physical Chemistry C 123, 8018 (2019)